Chapter 3: Nerve Cell Physiology
2nd edition as of August 2022
Chapter Overview
Now that you have a sense of the nervous system, it is time to examine the individual cells that comprise the nervous system. This might seem less important than understanding large structures in the brain, but drugs affect the body on the cellular level. Understanding how cells in the nervous system work is the first step toward comprehending why various drugs cause different psychoactive effects.
Here, we will examine the types of cells found in the nervous system and how an individual nerve cell conducts a signal. We will examine the different properties of this signal as well as study how signals jump from one cell to the next. Some basic knowledge of biology and chemistry is required to understand this chapter so it may help to review if you feel rusty.
Chapter Outline
- 3.1.1. Neurons: Signal Transmission Cells
- 3.1.2. Glia: Neuron Support Cells
- 3.2.1. Polarity of the Nerve Membrane
- 3.2.2. Conducting Electrical Signals: The Action Potential
- 3.2.3. Postsynaptic Potentials
Chapter Learning Outcomes
- Describe the structure and function of the neuron and its support cell.
- Describe how nerve conduction occurs.
3.1. Nerve Cell Structure
Section Learning Objectives
- Describe the parts of a typical neuron and their function, including the soma, dendrites, axons, and axon terminals.
- Describe glial and Schwann cells and explain their role in the formation of the myelin sheath.
- Explain the concept and importance of saltatory conduction.
- Distinguish between white matter and grey matter.
All tissues and organs in your body are made up of cells. Each cell has a nucleus that contains genetic data and is surrounded by a cell membrane. The membrane is important because it allows the cell to control what comes in and what goes out. The interior of the cell contains a number of different organelles, including mitochondria that produce energy in the form of adenosine triphosphate (ATP), ribosomes where proteins are produced, and lysosomes that contain proteins capable of breaking down proteins, lipids, and carbohydrates. To survive, cells must take in nutrients from outside the cell while removing waste that builds up inside itself. Neurons, more than any other cell in our body, must keep the inside of the neuron different from the outside and control the movement of substances across the membrane.
3.1.1. Neurons: Signal Transmission Cells
In the previous chapter, we mentioned that nerve fibers emanate from nerve cells called neurons or brain cells, which are responsible for generating and carrying electric signals throughout your body. Neurons are present everywhere in the nervous system—the brain, spinal cord, and periphery; nerves or nerve fibers are all made up of axons of the neurons. Neurons have a unique structure that helps them do this. Examine the following diagram:
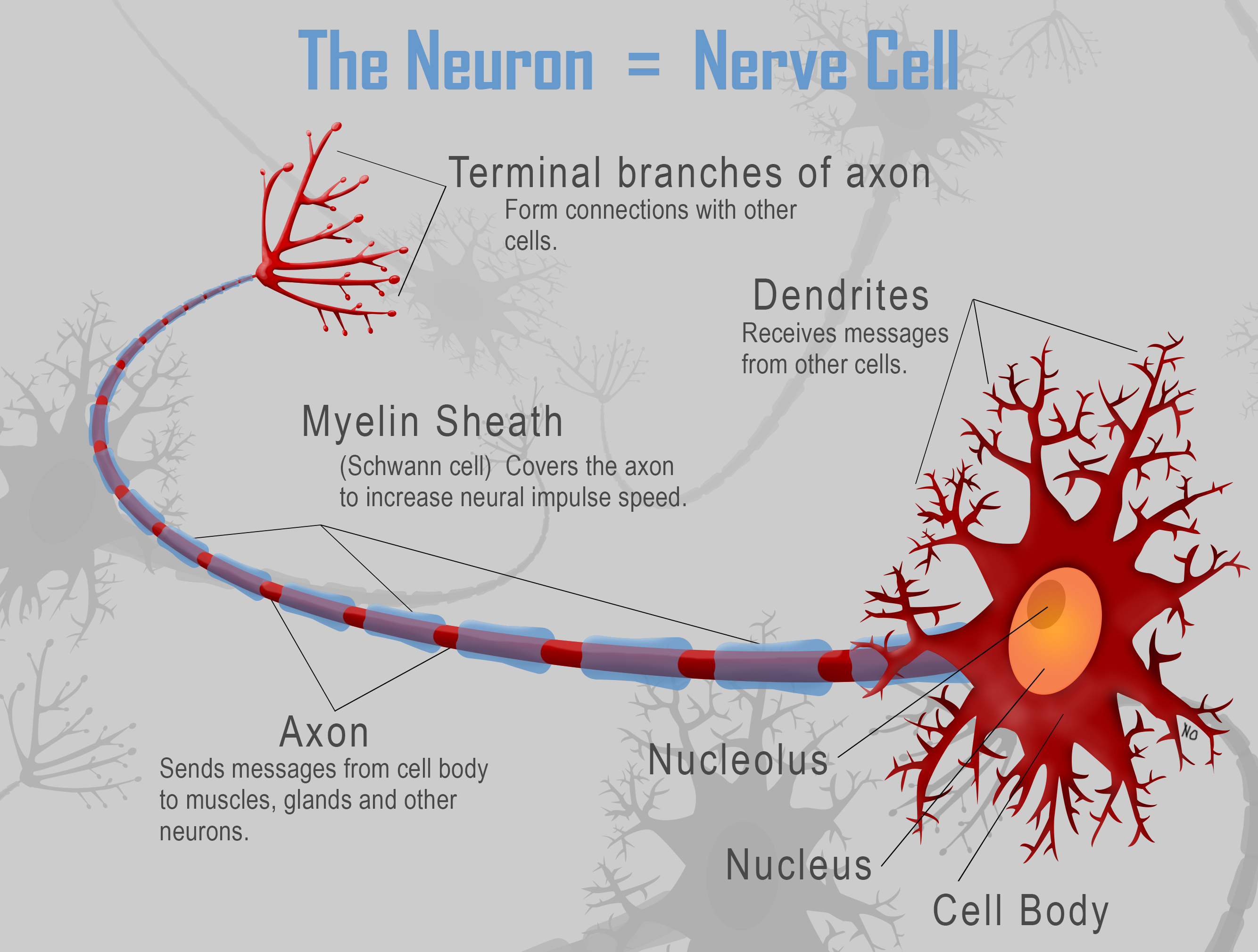
Figure 3.1. A Representative Neuron.
Original drawing by Nathan Olivier.
Here you can see the cell body or soma on the right. (Recall that soma is from the Greek for “body, which is how we got the term somatic nervous system.) The soma branches off into multiple dendrites that are responsible for receiving signals from other neurons. The name comes from the Greek word déndron for “tree” reflecting their tree-like shape and branches. Projecting from the some to the left is the axon, a long fiber that will communicate with other neurons. Each neuron can have many dendrites but only a single axon; signals are usually received by the dendrites, passed through the soma, and sent down the axon. The end of the axon splits into many branches or nerve terminals. It is here that the signal will be passed on to other cells by means of neurochemical transmission.
Neurons in the CNS connect to other neurons in the brain and spinal cord, while neurons in the PNS carry signals to and from various peripheral tissues. The length of the axon depends on the location of the neuron—axons can range from a thousandth of a millimeter to over a meter in some of the longest nerves in the human body, i.e., from the spinal cord to the lower extremities. Because some axons can be so long, the electrical signal must travel quickly down the length of the axon. Consequently, axons are wrapped in a myelin sheath not unlike the insulation around an electrical wire. To understand the myelin sheath, however, we will need to address the types of non-neuronal cells in the nervous system.
3.1.2. Glia: Neuron Support Cells
Neurons serve a critical role in the nervous system, but in order to function properly, they need the support of other cells, called glia or neuroglia. Many types of glial cells serve different functions, but you won’t need to name all of them for this class. Some provide physical support and direct neuron growth. Others provide neurons with nutrition, clear away waste, and maintain the environment around the neuron. Some even monitor for threats like our immune cells do in the rest of our body.
Two important types of glia that you need to be familiar with are Schwann cells and oligodendrocytes. Schwann cells wrap around axons in the PNS and form the myelin sheath mentioned above; their counterparts in the CNS are oligodendrocytes. They can also help nerves regenerate by removing damaged parts of the axon and guiding regrowth and neurogenesis. These cells do not continuously wrap around the entire axon. Between individual sections of the myelin sheath, there are small gaps where the axon is exposed. These gaps are called nodes of Ranvier and are very important for signal conduction along the axon. Keep this in mind during the next section, where we will discuss how the nerve conducts signals in the first place.
Are axons covered in myelin sheaths in the CNS?
You may have noticed in the previous section that Schwann cells are limited to the periphery. Despite this, axons in the brain and spinal cord are also covered in myelin. Myelinated nerve fibers in the brain constitute the areas that are called white matter. The name comes from the fatty content of myelin, which appears white after preservation. In comparison, gray matter contains more unmyelinated axons and appears darker as a result. You can see the difference in the picture of a dissected brain below. In the cerebral cortex and cerebellum, the gray matter is found in the outermost layer, while the white matter is located underneath. Gray matter makes up roughly 40%% of the brain, while gray matter constitutes 60%% of the brain.
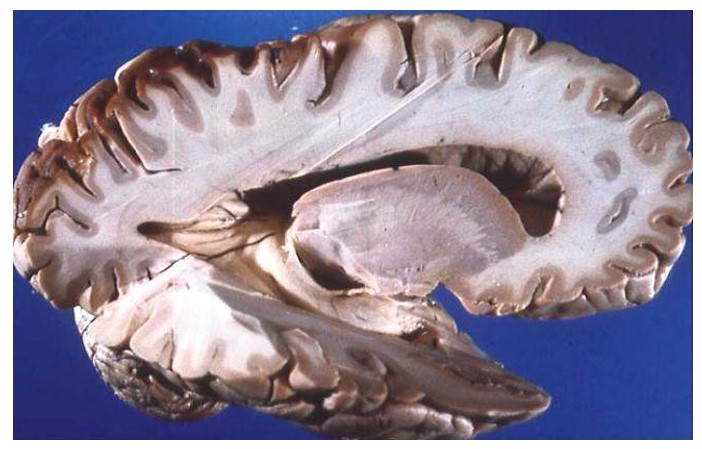
Figure 3.2. Gray Matter vs. White Matter. Source: Beal (2005). Photograph is licensed under the Creative Commons Attribution-Share Alike 4.0 International license.
The glial cells that produce myelin sheaths in the CNS are called oligodendrocytes. There are differences between oligodendrocytes in the CNS and the Schwann cells in the PNS. Unlike Schwann cells, which can only wrap around a single axon, oligodendrocytes can extend and wrap around multiple axons at once (see image below). They also cannot help axons regrow the way Schwann cells can, which is why even mild brain or spinal cord damage can be very dangerous and cause irreversible harm.
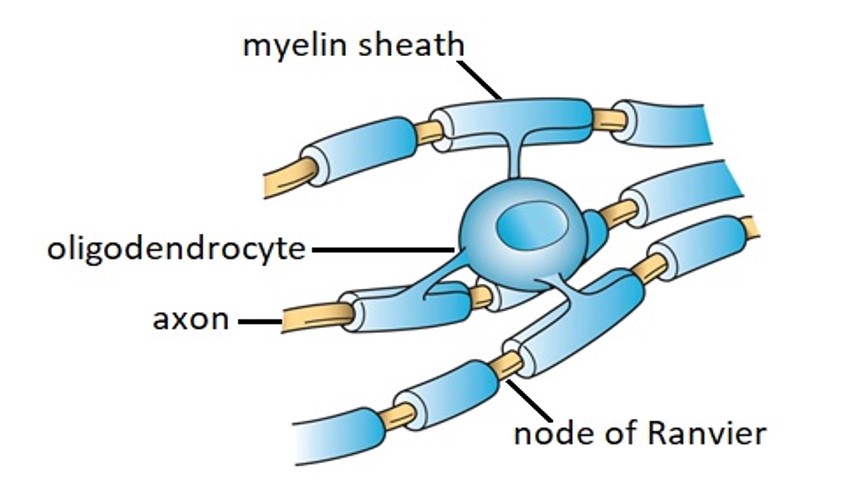
Figure 3.3. Oligodendrocyte Myelination of Multiple Nerve Fibers. Source: Fischer (2013). Figure was relabeled and is licensed under the Creative Commons Attribution-Share Alike 4.0 International license.
Glial cells are the most populous type of cell in the nervous system. Originally thought to play only a passive supporting role, they have only recently begun to be studied in depth. Their importance in brain function is increasingly recognized. Although we will not focus on them in this course, glial cells may play important roles in glucose storage and metabolism, saltatory conduction, and learning-related plasticity. If you are interested in learning more about their potential, you may read this article from National Public Radio about the important role of glial cells in the brain. Dysfunction of various glial cells has been implicated in various neurodegenerative disorders like Alzheimer’s disease, Parkinson’s disease, amyotrophic lateral sclerosis (ALS), and epilepsy.
Section Learning Objectives
- Explain how neurons are polarized and describe the state of a neuron at rest.
- Describe the action potential and define resting potential, threshold potential, depolarization, hyperpolarization, and refractory period.
- Define saltatory conduction and explain what causes it.
- Explain what a synapse is and provide examples of types of synapses.
- Define excitatory and inhibitory postsynaptic potentials.
To this point, we have consistently described neurons and nerves as capable of carrying signals around the body. You may have been able to imagine nerves as long wires that carry electrical signals, but this analogy can only get you so far. After all, neurons are not made of metal. If they were, we would always set off metal detectors. So, what exactly are these signals, and how are neurons able to create and conduct them? These are the questions we will be answering.
3.2.1. Polarity of the Nerve Membrane
Recall that at the start of the previous section, we mentioned how neurons, like all cells, must keep their cell interior different from the exterior. This is very important for neurons because they work electrically and need to conduct electrical signals. Neurons are only able to do this because they are polarized. The electrical charge across the cell membrane is unbalanced. In other words, the charge inside the cell is different than the charge outside the cell. Neurons accomplish this by using chemical ions which are atoms that have an electrical charge. Watch the following video to see how this works in action:
Membrane Potential (2-Minute Neuroscience [2:01]
Hopefully, the video helped you visualize and understand why neurons are polarized. Let us go over the important details here. Neurons have a resting potential of -70 mV, meaning they are more negatively charged inside the cell than outside the cell. They also keep two types of positive ions in a gradient across the membrane—sodium ions (Na+) want to get into the cell, while potassium ions (K+) want to get out. To remember the state of a neuron at rest, use the acronym INK: Inside the cell, Negative charge, K (potassium). This resting state is maintained by the neuron, which constantly consumes energy pumping sodium out of the cell and potassium in to maintain the gradient. This is due to the activity of the sodium/potassium pump (Na+/K+ pump) which preserves the differences in sodium and potassium concentrations on either side of the neuronal membrane.
3.2.2. Conducting Electrical Signals: The Action Potential
What happens when a neuron is stimulated and directed to fire? In most cases, stimulation causes the sodium gate at the mouth of sodium ion channels to open. There is much more sodium outside the neuron than inside. Consequently, positively charged sodium ions flow in, and cause the inside of the cell to become less negative. This decrease in charge is called depolarization because the neuron is less polarized than before, i.e., the membrane potential becomes less negative.
If the excitatory stimulus is sufficient, more sodium ions flow into the neuron, and the membrane potential reaches a critical value that is called the threshold potential. In most neurons, this is at about -55 mV. This value is important because there are additional ion channels that will open once this voltage is reached. They are fittingly called voltage-gated ion channels, and once they are opened, there is no going back. The neuron has now entered a runaway process that will result in an action potential—a reversal of the polarity that will travel across the neuron. This is the signal we have been referring to. To see it in action, watch this video explaining it (start at the 2:24 mark):
Neuron Action Potential (Made Easy) [3:24]
Now that you know how the polarity changes during an action potential, let’s examine the different terms and components of the action potential.
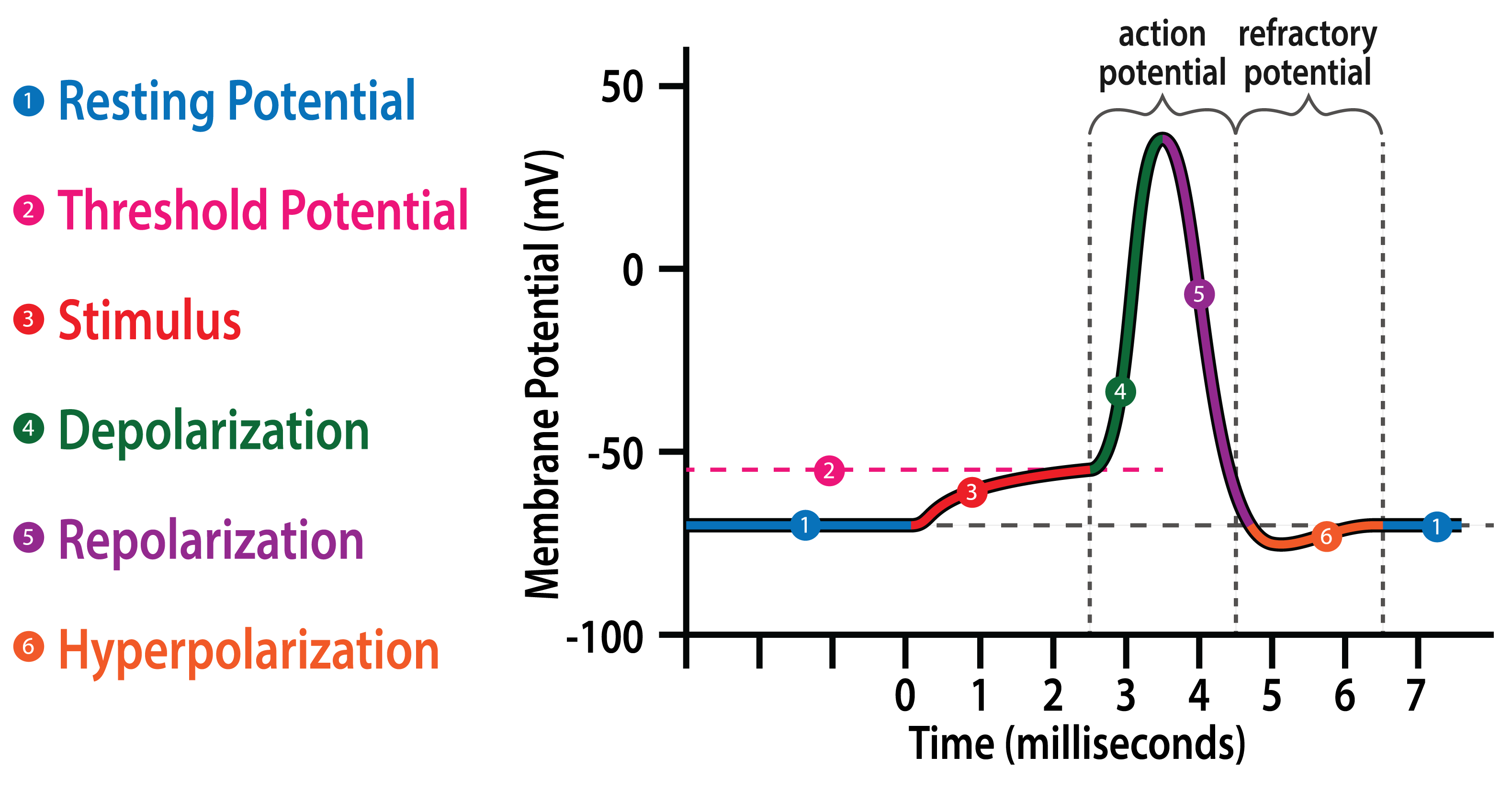
Figure 3.4. The Action Potential and Refractory Potential.
Original Drawing by Nathan Olivier.
We have already covered the resting potential and threshold potential, which are at -70 and -55 mV, respectively. Once the threshold potential is reached, voltage-gated ion channels open, and the neuron undergoes a rapid depolarization and repolarization, which is a return towards polarity. Together, the depolarization and repolarization constitute the action potential shown in the figure above. The repolarization overshoots, sending the charge past the resting potential and making the neuron hyperpolarized. When the neuron is hyperpolarized, the resting membrane potential is farther away from the threshold potential, and it is much harder to bring the neuron back to the threshold potential to trigger the action potential. Therefore, the period after an action potential is considered a refractory period. The absolute refractory period coincides with the action potential in the above figure when the membrane and it is impossible to re-stimulate the membrane to cause another depolarization. On the other hand, the relative refractory period coincides with the refractory potential when it requires a much stronger stimulus to excite the membrane. The refractory period is what causes the conduction of the action potential to travel in only one direction (unidirectional conduction).
As explained in the video, the action potential starts in the soma near the base of the axon because there are many voltage-gated ion channels in this area. The impulse then travels along the axon, opening the voltage-gated ion channels and reversing the polarity in successive sections of the axon.
To speed up this process, some axons are covered in myelin sheaths which insulate the axon and prevent ions from crossing the membrane. How does this speed up the impulse? Recall that the sheath is made up of many individual Schwann cells and that there are small gaps called nodes of Ranvier between them. Action potentials can only be triggered at these exposed nodes, so the impulse “leapfrogs” rapidly from node to node. This process is called saltatory conduction and is why myelinated axons conduct signals faster than unmyelinated ones. Check out this short video to see it animated:
3.2.3. Postsynaptic Potentials
Eventually, the nerve impulse travels all the way to the end of the neuron at the axon terminal. How does the neuron transfer the signal to another cell? If the axon terminal were touching the other cell, it could transfer ions directly, continuing the impulse. While these types of connections are possible, they are very rare in humans. Instead, there tends to be a gap between the axon terminal and the receiving cell. This gap and its surrounding structures make up a synapse, and we can call the receiving cell the postsynaptic cell. Most synapses connect axon terminals to dendrites (called axo-dendritic), but other axons connect to the soma (axo-somatic) or to another axon (axo-axonic) as shown in the illustration below.
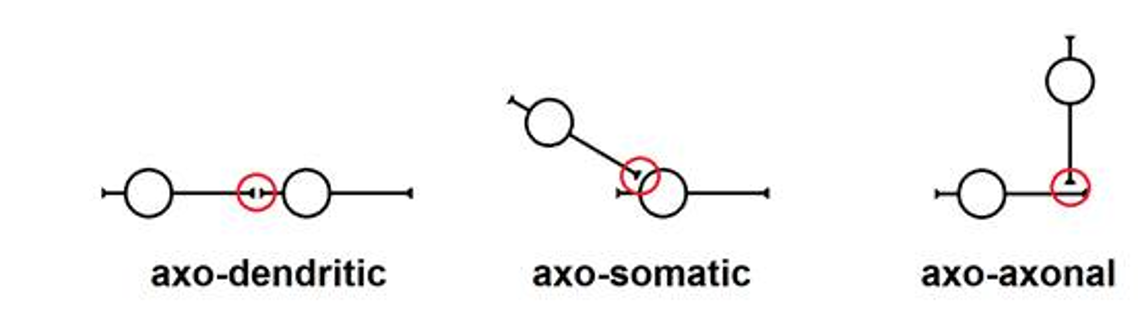
Figure 3.5. Different Types of Neuronal Synapses.
Original drawing by Raymond M. Quock
When the action potential reaches the axon terminal, it causes the terminal to release certain neurochemicals called neurotransmitters into the synapse. These chemicals can bind to receptors on the postsynaptic cell, opening or closing various ion channels. More detail will be covered in the next chapter. For the time being, it is important to know that some of those chemicals can increase the polarity of the postsynaptic cell, while others can decrease the polarity. This is what determines the excitatory or inhibitory effects of neurotransmitters. The effect depends on the neurotransmitter released by the axon terminal, as well as the types of receptors on the postsynaptic cell. This will determine whether the neurotransmitter produces an excitatory or inhibitory effect on the neuron.
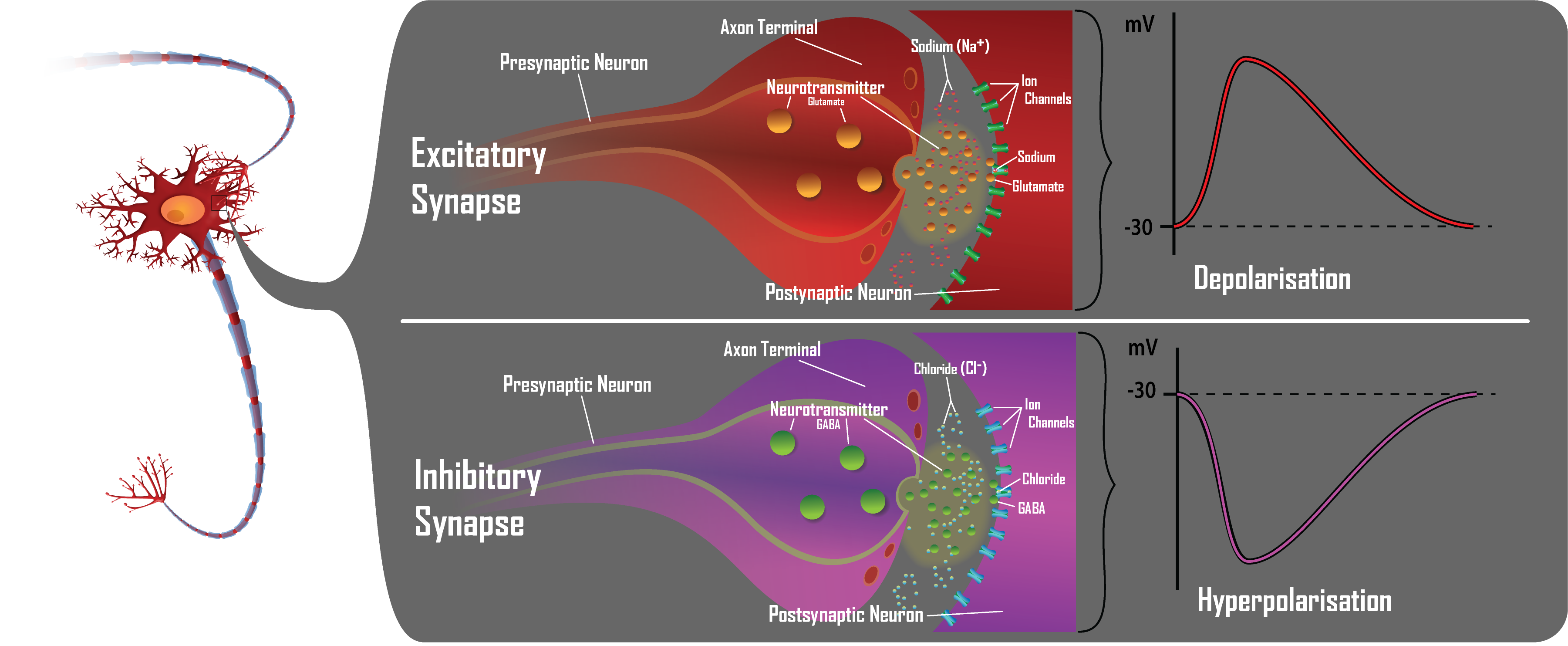
Figure 3.6. Excitatory and Inhibitory Effects of Glutamate and GABA.
Original drawing by Nathan Olivier.
Take the above image to illustrate this point. In the top panel, the axon terminal releases the excitatory neurotransmitter glutamate (GLU), which causes sodium ion channels to open in the postsynaptic neuron. This causes it to depolarize, bringing it closer to the threshold potential. This is called an excitatory postsynaptic potential (EPSP) because it “excites” the postsynaptic neuron or makes it easier for it to fire. By comparison, the axon terminal in the lower panel releases the inhibitory neurotransmitter gamma-aminobutyric acid (GABA), which opens chloride ion channels in the neuron. Since chloride ions are negatively charged, this causes a hyperpolarization making the inside of the neuron become even more negative, moving it farther away from the threshold potential. This is called an inhibitory postsynaptic potential (IPSP) because it inhibits the postsynaptic neuron and makes it less likely to fire.
A single EPSP is generally insufficient to cause the postsynaptic neuron to fire. Yet, a single neuron can receive multiple excitatory signals from numerous other neurons, and each excitatory signal contributes an EPSP. In this way, the cumulative EPSPs can add up to reach the threshold potential, as seen in the image below:
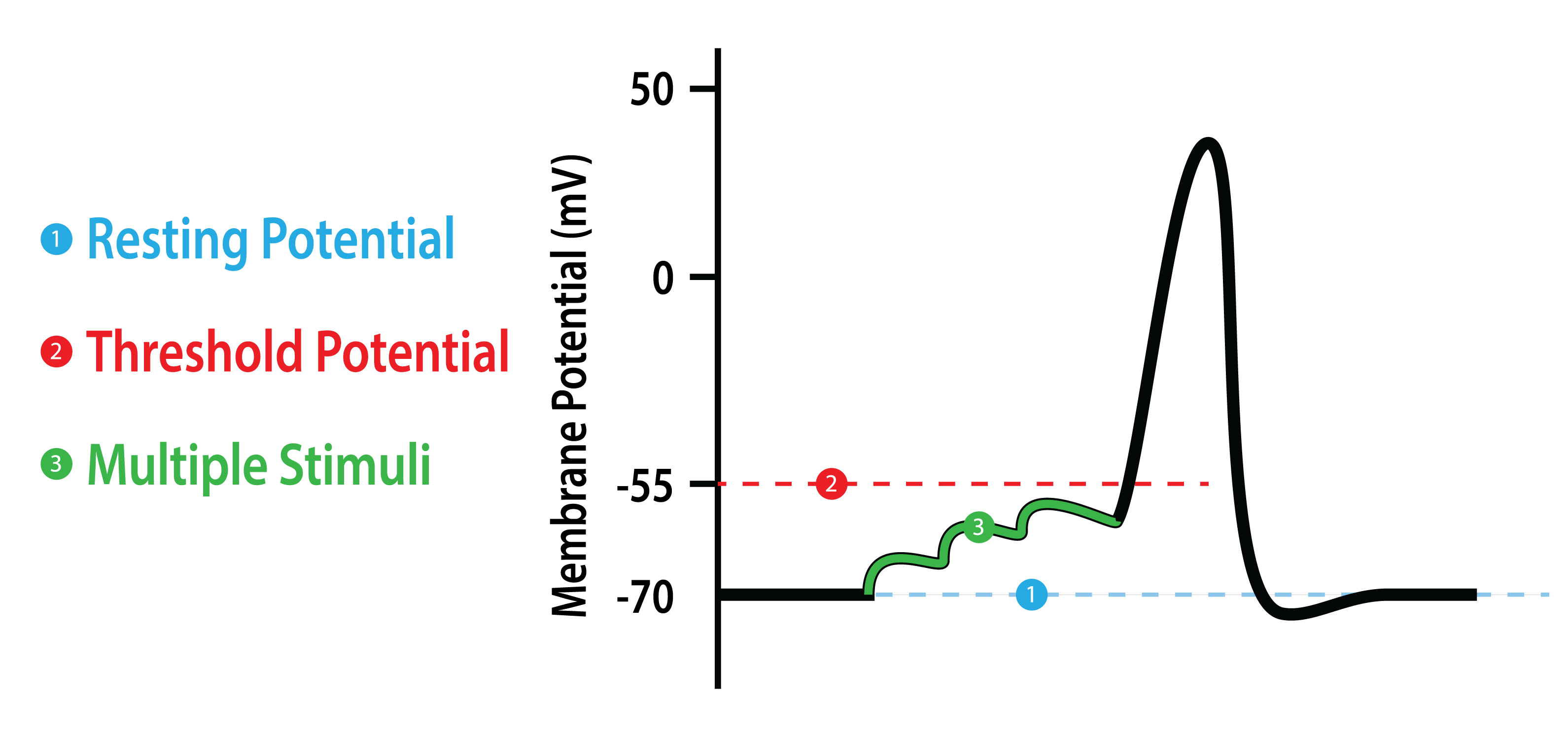
Figure 3.7. Multiple EPSPs and the Threshold Potential.
Original drawing by Nathan Olivier.
A single neuron may have postsynaptic receptors that respond to neurotransmitters released from hundreds of axon terminals, any number of which may be sending excitatory or inhibitory signals to the neuron. This complex interaction is what drives all of the integration, processing, and coordination that occurs in your brain.
That is all for this chapter. Make sure you understand this chapter before moving on to the next since we will be covering how neurons communicate with each other across the synapse in detail and will be using a lot of the terminology and concepts established in this chapter. Ask your instructor for help if you need it.
Chapter Summary and Review
In this chapter, we took a closer look at the individual cells that make up the nervous system: the neurons that are responsible for transmitting signals; and the glia cells that support the neurons. We then thoroughly explored how changes in the membrane permeability to chemical ions contribute to the membrane potential and generation of an action potential. Conduction of an electrical signal entails reversal of the polarity in successive sections of the nerve membrane. We also saw how the myelin sheath increases the speed of conduction of an action potential along a nerve axon. Electrical signals trigger the release of neurotransmitters into the synapses that separate neurons and other tissues, and excitatory and inhibitory postsynaptic potentials determine the response of the receiving cell. Make sure you understand this chapter before moving on to the next. We will be covering how neurons communicate with each other over the synapse in detail and will be using a lot of the terminology and concepts established in this chapter. Check your understanding of the material with the practice questions and ask your instructor for help if you need it.
Chapter 3 Practice Questions
Answer the following questions.
- Draw a picture of a neuron and label the soma, dendrites, axon, and axon terminals.
- What types of cells are Schwann cells and oligodendrocytes? Where are they located, and how do the two differ?
- What is the difference between white matter and grey matter?
- State whether each of these ions is positively or negatively charged: sodium, potassium, and chloride.
- What does it mean when a nerve membrane is polarized?
- What maintains the polarization of the nerve membrane?
- What is the typical electrical charge of a neuron at rest? Give the answer in millivolts (mV).
- Explain depolarization and hyperpolarization. Which makes it easier for a neuron to fire?
- How do membrane potentials achieve the threshold potential?
- How does reaching the threshold potential trigger creation of an electrical potential?
- What are the different phases of the action potential?
- Explain how saltatory conduction works.
- What is the difference between myelinated and non-myelinated nerve fibers in terms of conduction speed?
- What are the two types of postsynaptic potentials? Which causes depolarization, and which causes hyperpolarization?
2nd edition